The future of wild blueberries: Testing warming impacts using open-top chambers
By Rafa Tasnim 1,‡, Lily Calderwood 2, Seanna Annis 3, Francis Drummond 4, Yong-Jiang Zhang 5,‡
1 Graduate Student and Research Assistant, School of Biology and Ecology, University of Maine, Orono. Contact: rafa.tasnim@maine.edu
2 Extension Wild Blueberry Specialist and Assistant Professor of Horticulture, School of Food and Agriculture, University of Maine, Orono, ME. Contact: lily.calderwood@maine.edu
3 Associate Professor of Mycology, School of Biology and Ecology, University of Maine, Orono, ME. Contact: sannis@maine.edu
4 Professor of Insect Ecology and Insect Pest Management and Blueberry Extension Pollination Specialist, School of Biology and Ecology, University of Maine, Orono, ME. Contact: fdrummond@maine.edu
5 Assistant Professor of Plant Physiology, School of Biology and Ecology, University of Maine, Orono, ME. Contact: yongjiang.zhang@maine.edu
‡Corresponding Authors. Email: rafa.tasnim@maine.edu, yongjiang.zhang@maine.edu
ABSTRACT
Wild blueberries are one of the most economically and culturally important crops in Maine. Global climate change has already changed the growth pattern of crops in this region. However, it is not known how wild blueberries (Vaccinium angustifolium) will respond to warming. Here we present the methodology we used to investigate warming effects on wild blueberries and preliminary results to foster interdisciplinary and collaborative research. A 2.6 m2 open-top chamber (OTC) was designed and built to accommodate wild blueberry plants in the field. In order to test the most effective method of heating the chamber, both passive and active heating was tested. The active heating system consistently maintained air temperature 3 to 5ºC higher than the ambient control. The passive heating system increased the temperature during the day but decreased the temperature at night. Under the active heating treatment, the photosynthetic rate and water-use efficiency of the wild blueberry plants decreased. Plants also produced smaller and thinner leaves with lower leaf mass per area under active heating compared to the control (ambient temperature) treatment. Moreover, whole plant CO2 assimilation rate also decreased (32% to 40% lower) under warming. These preliminary results indicate that warming had a negative effect on both leaf and plant level performance of wild blueberries. While these results need to be tested on a larger scale and over longer terms, we suggest the importance of studying climate change effects on crops. We also suggest actively heated OTC as a sound method of studying ground-level response to warming. This tool could be used for further studies on the responses of wild blueberries and other crops to warming in Maine. This information is crucial for developing techniques and policies that allow farmers to adapt to climate change.
Introduction
Anthropogenic climate change brings relatively quick changes in climate conditions and increased climate variability, which pose great challenges to worldwide agricultural systems (Bita & Gerats 2013). Atmospheric temperatures have increased significantly in the past century and will continue to climb in the future because of greenhouse gas emission. Atmospheric temperatures are predicted to increase by 1.8 to 4ºC at the end of this century (IPCC 2014). In Maine, USA, temperatures are expected to increase 2 to 6ºC more by the end of this century (The University of Maine Climate Institute 2015). Global warming will significantly influence the physiology, growth, and yield of crops since crop production may be stimulated by 1 to 3ºC increases in temperate regions whereas crop production might be hindered by any degree of warming in tropical and subtropical regions (Easterling et al. 2007; Hatfield et al. 2011). Different plants and ecosystems have distinct responses to warming with different ranges of temperatures for physiological performance and growth (Hatfield et al. 2011; Zhang et al. 2016). Both positive and negative effects of warming on crop yield have been reported (Easterling et al. 2007; Hatfield et al. 2011; Semenov & Shewry 2011), but it is still unclear how the physiological performances of temperate crops, such as Maine’s wild blueberry, will respond to warming.
Warmer temperatures due to climate change affect the development of temperate fruits and vegetables by impacting various plant processes such as photosynthesis, respiration, and water and nutrient transport (Magan et al. 2011). Warming has been found to have negative effects on a variety of temperate crops (Lobell & Field 2007). For example, temperate crops such as maize and wheat show increasing leaf photosynthetic rates due to increasing temperature up to 33ºC and lower final biomass (Reynolds et al. 2000; Badaruddin et al. 1999). Reynolds et al. (2000) also observed lower chlorophyll content and total canopy photosynthetic rate in temperate crops under warmer environments.
However, under controlled warming experiments on temperate crops, studies from various regions reported contradictory observations under different warming temperatures. Mitchell et al. (1995) reported that winter wheat phytomass and yield declined by 20 to 30% when ambient temperature was warmed by 4ºC. Xiao et al. (2010) reported that winter wheat yields increased by 6% when ambient temperature was warmed by 2.2ºC, whereas, Fang et al. (2010) reported that winter wheat yields declined by 27% when ambient temperature was warmed by 2.5ºC. To our knowledge, the effects that future warming will have on small fruit crops, specifically wild blueberry (Vaccinium angustifolium), and other crops in Maine, USA has not been studied.
Wild blueberry production is a unique semi-natural agricultural system. Wild blueberry plants are initially established from seeds naturally (not planted) and then underground stems (rhizomes) develop (Fig. 1). Roots then grow directly off of rhizomes creating a tightly woven mat across fields. Rhizomes grow within approximately 10 cm of the soil surface and produce upright stems above the soil surface. An individual wild blueberry plant, with its spreading rhizome system, is referred to as a genet (Bell et al. 2009) (Fig. 1). Each genet is genetically different from neighboring plants which together create a complex mixture of genotypes in the wild blueberry field providing natural resilience to pests and a rich diversity of flavors to consumers.
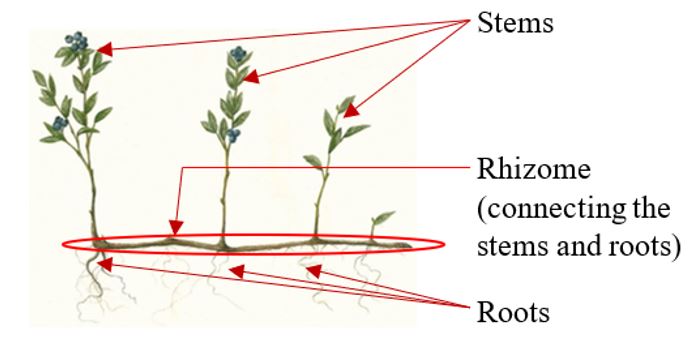
Here, we present the methodology used to manipulate warming in the field using open-top chambers and preliminary wild blueberry plant response to 3 to 5°C warming inside the chambers. We used a representative wild blueberry genet to address the following questions: 1) Is passive or active heating the most effective way to heat an open-top chamber to study warming effects on the wild blueberry crop? and 2) Will warming enhance or reduce physiological and growth performances of wild blueberry? A systematic approach was used to study the response of morphological, structural, and physiological processes to warming. We sought to establish the mechanistic linkages among different physiological processes including water use, nutrition, and carbon assimilation using a representative genet, which will be tested further with a larger and long-term experiment including more genets. Anyone interested in using our system to study climate change impacts on crops, pests, micro-organisms, and human nutrition could contact the authors.
Materials and Methods
Experimental Site
The study site was located at the Blueberry Hill Farm in Jonesboro (Longitude: -67.6495° N, Latitude: 44.6454° W), Maine, USA which is the only university-based (The University of Maine) wild blueberry research facility in the United States. The average annual temperature in Jonesboro, Maine is 6.3ºC with an annual low monthly average temperature of 0.9ºC and annual high of 11.7ºC. The average annual precipitation and snowfall are 1298 mm and 158 cm (Climate data for Jonesboro, Longitude: -67.6495° N, Latitude: 44.6454° W; average weather Jonesboro, ME – 4648 – 1981-2010 normal). The experiment was conducted in a field that was in the crop cycle (second year cycle) in 2018. Wild blueberries are managed on a two-year cycle: during the first year the plants are pruned and grow vegetatively and in the second year the plants flower and produce a fruit crop. The soil at the site is classified as a Colton gravelly sandy loam which is excessively drained and has a depth to water table of greater than 203 cm (Hunt et al. 2008). The experiment was conducted from June to September 2018. During this period, no additional irrigation or fertilizer was applied in the experimental field. Notably, only one large representative genet (~10 m2) was selected to test the chamber system and conduct the preliminary trial.
Chamber Design
Three areas were selected within the same wild blueberry genet to compare among three different treatments: one area was warmed using the Open-Top Chamber (OTC)-cable system (Fig. 2a & 2b) which is indicated as AH (Active heating) treatment. The OTC was used in our warming study because among different types of heating system, the OTC was proved to be an energy and cost- efficient one which can increase both air and soil temperature concurrently representing the actual global warming concept (Sun et al. 2013). The OTC-cable system with a 288 W power rating consisted of a standard International Tundra Experiment (ITEX) six-sided OTC (Bokhorst et al. 2008), an RKF Series Silicone Heating Tape, and a heat resistant PEX-tube. The design of the OTC and heating system followed Sun et al. (2013). The OTC was constructed with a LEXAN polycarbonate sheet (glass substitute) of the following dimensions: 3 mm thickness with a 100 cm base, 70 cm top, and 55 cm sides cut at an angle of 60°. These sheets feature high light transmittance (86%) and high infrared transmittance (~85%). A 12 m waterproof silicone heating tape with an 20 Wm-1 and 240 W power rating (Briskheat, Columbus, OH, USA) was coiled around a heat resistant PEX tubing (2.5 m in length and 3.5 cm in diameter), after which the tube was reshaped into a circle, wrapped with an aluminum tape, and fixed inside the OTC (using timber stakes) at a height of 15 cm. PEX tubing distance to plants was 10 to 15 cm while distance to OTC inner walls was 15 cm. This was to prevent compromising the OTC or risk burning the plants. Another OTC without the heating tape system was installed in a second area (Fig. 2c) and represented the Passive heating (PH) treatment. The third area (Fig. 2d) was staked out as a control treatment (NC) under the ambient weather condition with no chamber or heating system installed.
Environmental Variables
Atmospheric temperature and relative humidity (Fig. 3) were instantaneously recorded every 10 minutes using Watchdog 1000 series micro stations (Spectrum Technologies, Inc, Aurora, IL, USA) in all three area from June 2018 to September 2018. Vapor pressure deficit in air was calculated using the atmospheric temperature and relative humidity data. Soil temperature and volumetric water content were measured by a Fieldscout TDR 150 Soil Moisture Meter (Spectrum Technologies, Inc, Aurora, IL, USA) from six random places within each of the treatment areas.
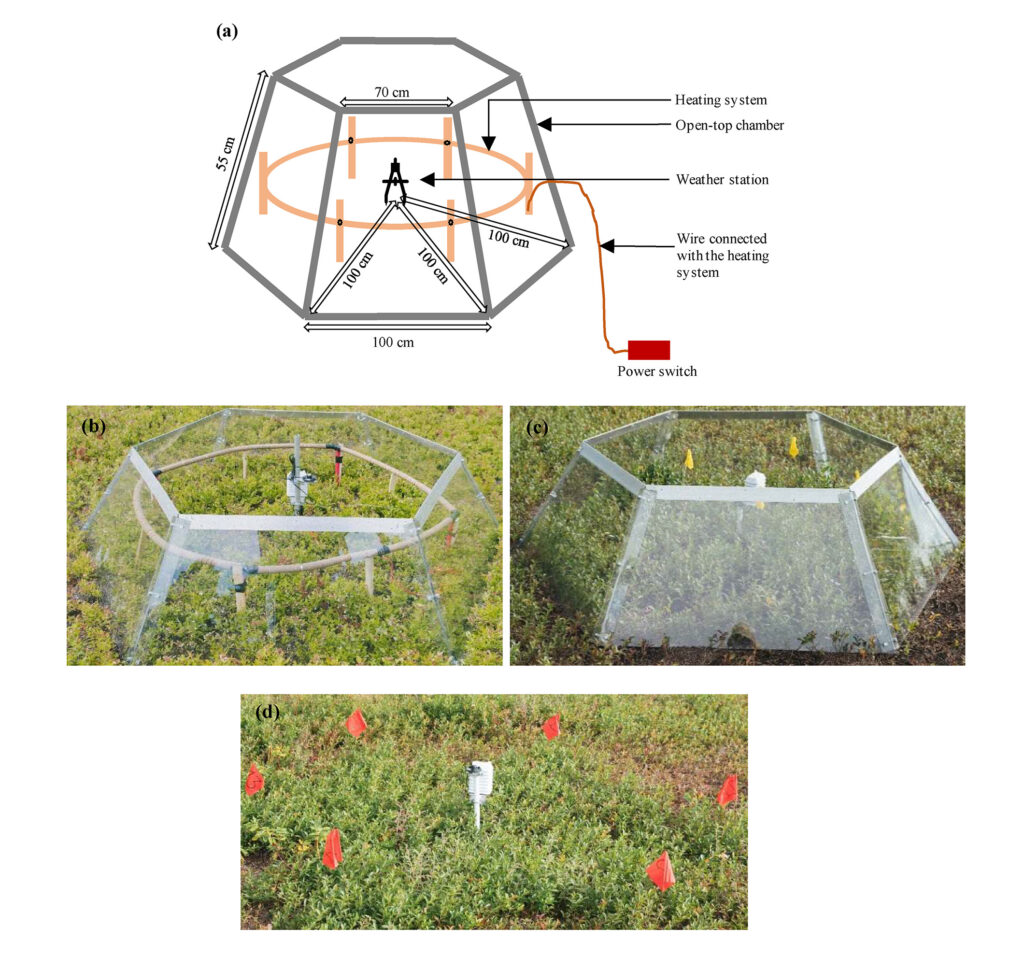
Leaf and stem structural traits
Six wild blueberry stems were randomly selected from each treatment plot and their leaf and stem structural traits (stem length and diameter, leaf number, leaf size, total leaf area and dry biomass) were measured. Samples were collected in August 2018 at the end of the growing season of wild blueberry plants. Leaf area was determined using a LI-3000A area meter (Li-Cor, Lincoln, NE, USA), then the leaves were oven-dried at 70ºC to constant mass and weighed. Leaf mass per area (LMA) was determined as leaf dry mass divided by leaf area (g m–2). Stem volume was measured using water displacement method (Archimedes’ principle) with a precision balance (0.0001gm). The stems were oven-dried at 70ºC to constant mass and weighed and the wood density was determined as stem dry mass divided by stem volume (g cm–3).
Leaf water status and Chlorophyll concentrations
Six wild blueberry stems were randomly selected from each treatment area to measure midday leaf water potential and chlorophyll content. Leaf water potential was measured by a leaf pressure chamber (PMS Inc., Albany, OR, USA) on samples collected at midday 12:30 h solar time. Chlorophyll content per leaf area was measured by a SPAD Chlorophyll Meter (SPAD 502; Minolta Corp., Osaka, Japan). Chlorophyll content per leaf mass was determined as chlorophyll content per leaf area divided by LMA.
Plant physiological traits
Leaf stomatal conductance, photosynthetic rate, and intercellular CO2 concentration from twelve randomly selected wild blueberry stems in each treatment area were measured by a portable photosynthetic measurement system (li-6400; Li-Cor Biosciences, Lincoln, NE, USA) on a typical sunny day (17 August 2018) between 11:00 and 14:00 h solar time at a photosynthetic photon flux density of 1500 µmol m–2 s–1. Pre-dawn Fv/Fm (maximum potential quantum efficiency of photosystem II) was measured from six randomly selected wild blueberry stems in each treatment area by a portable photosynthetic measurement system (li-6400; Li-Cor Biosciences, Lincoln, NE, USA). During the measurements, the atmospheric temperatures, relative humidity and leaf temperatures ranged from 25ºC to 30ºC, 50% to 60%, and 27 to 31ºC, respectively. Leaf photosynthetic rate per mass was determined as the photosynthetic rate divided by LMA. Water use efficiency of wild blueberry stems was determined as the photosynthetic rate divided by the stomatal conductance of leaves. CO2 assimilation rate per stem was determined as the photosynthetic rate (area based) multiplied by the total leaf area of an individual stem.
Leaf nutrient concentrations
Leaf nutrition was assessed by excising leaves from 12 randomly selected wild blueberry stems from each treatment. In order to get enough dry mass for nutrient concentration measurements, leaf samples from the 12 stems per treatment were pooled into two samples and sent to the University of Maine Soil and Plant Tissue Testing laboratory in Orono, Maine. One individual stem in treatment did not have enough dry mass for leaf nutrient concentration determinations.
Data Analyses
Statistical analyses were applied using SPSS V21 (IBM Corp., Armonk, NY, USA). ANOVA analyses followed by Tukey’s post-hoc HSD test were conducted to determine the statistical significance of the data among the three treatments (Control, Passive heating and Active heating) at a significance level of 0.05. Our assumption in using ANOVA in this non-replicated treatment experiment was that the between stem variance was as great as between treatment variance within the genet, and therefore used stems within treatments as replicates. In a heavily replicated experiment on leaf and flower node removal in lowbush blueberry, variance among stems within a genet was found to be as high as among treatment plots within genets for 50% of the measured physiological responses, suggesting that stems within a genet respond similarly whether they are spatially proximal or not, despite the rhizome structure underground that connects stems within a genet (Bajcz & Drummond 2017). This was true even when rhizomes were severed among stems. We found in a preliminary variance components analysis of our data with one morphological measure (LMA) and two physiological measures (chlorophyll concentration and photosynthetic rate) that in two of three measures the overall stem within genet variance was not different than variance among the locations within the genet. Only with LMA, the variance among locations within the genet was greater than the variance between stems within the genet. Therefore, our assumption holds for at least some of our measures. Since only one genet was used here and stems were the only basis of replication, the probability of a Type I error might be biased toward a low probability level.
Results
During June-September 2018 (Fig. 3a), in the passive heating (PH) and active heating (AH) treatments, the average temperature was approximately 0.5ºC and 3.5ºC higher, respectively, compared to the control (NC). Over the same period, the relative humidity (RH) (Fig. 3b) in the PH and NC treatment was not significantly different from each other but in the AH treatment it was 10% lower compared to the NC and PH treatments. The average air vapor pressure deficit (VPD) (Fig. 3c) in the NC and PH treatments was similar, 0.6 to 0.8 kPa. However, in the AH treatment, the VPD was 1.26 kPa which is 0.5 kPa higher compared to the NC and PH treatments.
On a typical sunny day (17 August 2018), the atmospheric temperature was 17.6 ± 0.3ºC, 18.1 ± 0.5ºC and 21.8 ± 0.4ºC in the NC, PH and AH treatments, respectively (Fig. 3d). On average, the temperature was approximately 0.5ºC higher and 4ºC higher in the PH and AH treatments, respectively, compared to the NC treatment. However, the air temperature was lower inside the PH chamber during the night than the ambient air temperature. On the same day, the atmospheric RH (Fig. 3e) in the NC and PH treatments was similar, 86.8 ± 1% and 87.3 ± 1.2%, respectively but in the AH treatment, the RH was 72.6 ± 0.7% which was 13% lower compared to the NC and PH treatments. Consequently, the VPD was 0.33 ± 0.03 kPa, 0.4 ± 0.04 kPa and 0.8 ± 0.04 kPa in the NC, PH and AH treatments, respectively (Fig. 3f). Based on the 0.5 to 4ºC higher atmospheric temperature and 13% lower RH, the VPD in AH treatment was approximately two times higher compared to the NC and PH treatments.
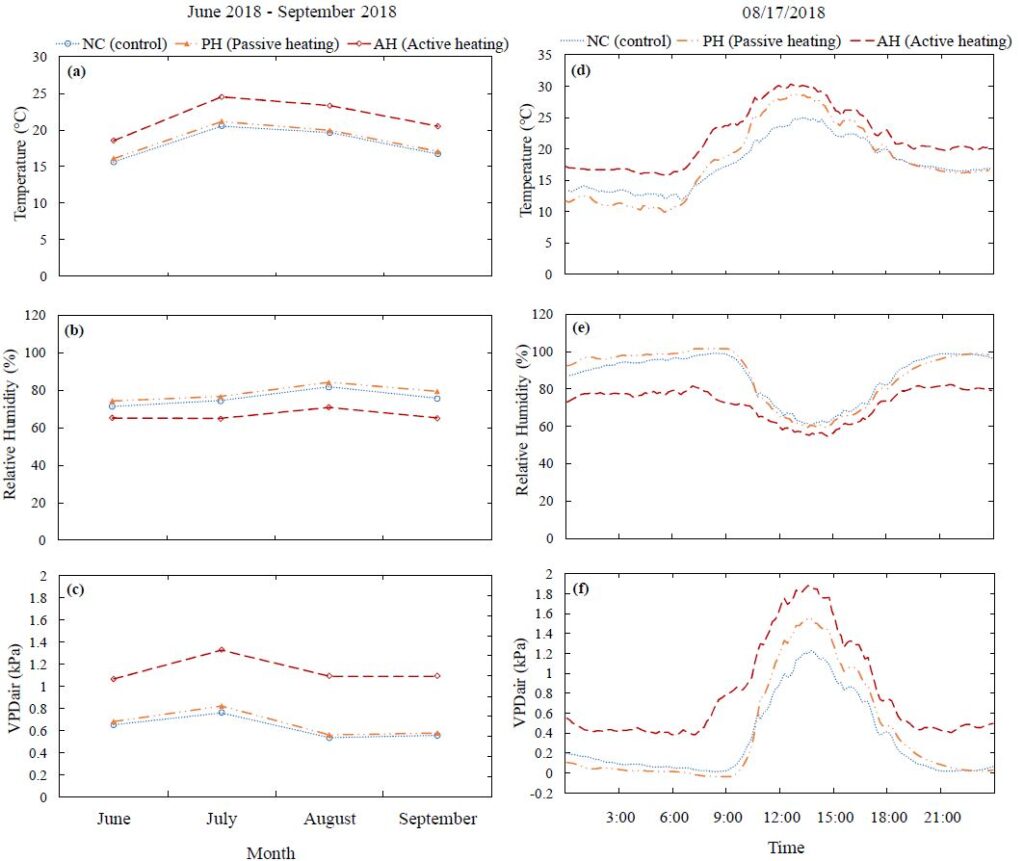
Soil temperature was significantly higher in the AH treatment compared to the other two treatments, which was expected. In contrast, soil temperature was slightly, but significantly lower in the PH treatment compared to the NC treatment (Fig. 4a). Consequently, the volumetric water content (VWC) in soil was significantly lower in the AH treatment compared to the NC and PH treatments (Fig. 4b). There was no significant difference between the NC and PH treatments in soil VWC. The average leaf water potential (LWP) (Fig. 4c) in the AH treatment was approximately 0.5 MPa lower than the LWP in the PH treatment and approximately 0.9 MPa lower than the control.

Photosynthetic rates per leaf area of wild blueberry stems were significantly lower in both PH and AH treatments compared to those of the control (Fig. 5a). However, the observed photosynthetic rate per leaf mass of wild blueberry stems was similar among the NC, PH and AH treatments (Fig. 5b). No significant difference in maximum quantum efficiency of Photosystem II of the wild blueberry stems (Fv/Fm) were detected among the NC, PH and AH treatments (Fig. 5c). Similarly, the observed stomatal conductance (Fig. 5d) of wild blueberry stems showed no significant differences among different treatments.
In accordance with the lower photosynthetic rate, the leaf intercellular CO2 concentration (Fig. 5e) of wild blueberry stems was significantly higher in AH treatment compared to the NC and PH treatments. The AH treatment also significantly decreased water use efficiency of wild blueberry stems compared to those of the NC but not significantly higher than that of the PH treatment (Fig. 5f). At a similar level of stomatal conductance for all three treatments (Fig. 5d), the photosynthetic rate per leaf area was significantly (Fig. 5a) lower for the PH and AH treatments compared to the control.

The PH treatment had significantly lower chlorophyll per leaf mass than the AH or NC treatments (Fig. 6a). Chlorophyll concentration per leaf area was significantly lower in PH and AH treatments (Fig. 6b) and had 20% lower total nitrogen concentration (Fig. 6c and 6d) compared to the NC treatment. This agrees with the observed lower photosynthetic rate per leaf area (Fig. 5a). In the AH treatment, a 40% higher phosphorous concentration per leaf mass was observed compared to the NC and PH treatments (Fig. 6e) whereas only a slight difference was observed in phosphorous concentration per leaf area (Fig. 6f) among the NC, PH, and AH treatments. However, no difference was observed in total carbon concentration per leaf mass among the treatments (Fig. 6g), whereas, a higher carbon concentration per leaf area was observed (Fig. 6h) in the NC treatment compared to those of the AH and PH treatments.


For the stem structural traits, stem length (Fig. 7a) and diameter (Fig. 7b) were significantly smaller in the PH and AH treatments compared to the control. In contrast, from the leaf structural traits of wild blueberry plants, it was observed that the number of leaves was significantly higher in the AH treatment compared to the control (Fig. 7c) whereas the leaf size of wild blueberry stems was significantly smaller in both heating treatments compared to the stems in the control (Fig. 7d). Hence it is observed that, with the increasing atmospheric temperature wild blueberry stems were developing more leaves with smaller surface area per leaf. The average total leaf area per stem was smaller in the heating treatments compared to the control (Fig. 7e), but the difference was not significant. Dry biomass of leaves (Fig. 7f) was lower (although not significant) and leaf mass per area (Fig. 7g) was significantly lower in PH and AH treatments compared to the control. In contrast, no significant difference was observed in wood density among the treatments (Fig. 7h). The CO2 assimilation rate per stem was significantly (32% to 40%) lower in the AH and PH treatments compared to the control (Fig. 8).
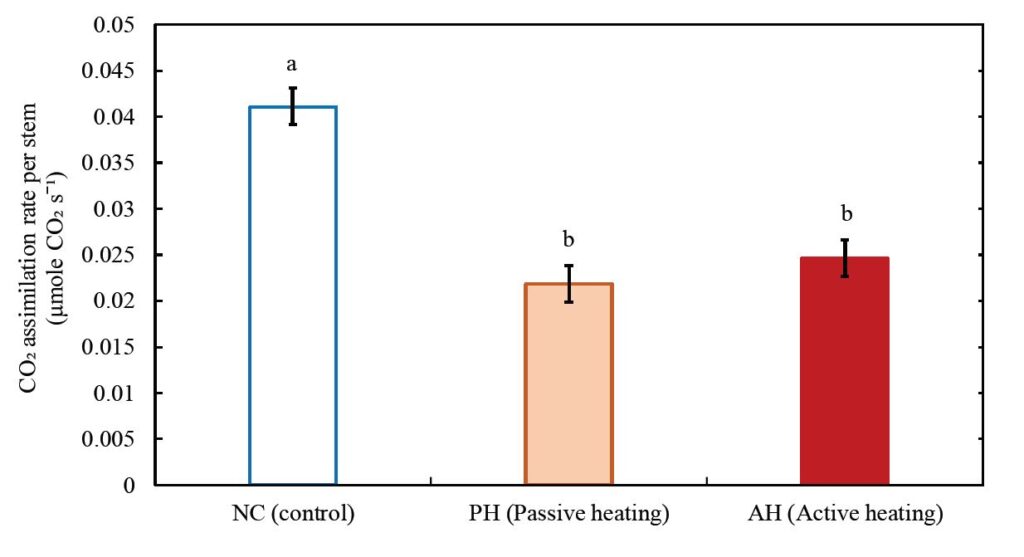
Discussion
Our results indicate that the open-top chamber (OTC) with active heating (AH) system is an efficient and feasible way to manipulate plant canopy and soil temperatures. We found that we were able to successfully measure warming effects on physiological development of wild blueberry plants in the field. The passive heating (PH) chamber produced warmer temperatures during the day, but cooler temperatures during the night inside the chambers. This pattern introduced uncertainties and confounding factors to the experimental design. By increasing temperatures of 3 to 5ºC, the AH chamber effectively altered plant morphology, structure, and physiology. Warming decreased leaf photosynthetic rates and water use efficiency of wild blueberries. Warming by both the AH and PH also decreased leaf sizes but increased total leaf numbers. The cost of building an AH chamber including the weather station for monitoring temperature, RH, and soil moisture was ~$1,800.
Many temperate crops are expected to benefit from 1 to 3ºC warming of ambient temperature (Easterling et al. 2007; Hatfield et al. 2011). Photosynthetic rate of plants generally is expected to increase with increasing temperature between 13ºC and 25ºC assuming that no other limiting factors such as light, nutrient, and CO2 concentration changes occur (Curtis & Clark 1950). Some temperate crops (i.e., wheat, maize) exhibit the maximum photosynthetic rate at 33ºC, but with photosynthesis declining after the temperature reaches more than 33ºC (Reynolds et al. 2000; Badaruddin et al. 1999). Forsyth & Hall (1965) observed that the rate of apparent photosynthesis in wild blueberry crops increased with increasing leaf temperature from 13ºC to 29.5ºC under laboratory conditions. However, in our study, photosynthesis of wild blueberry declined when air temperature increased to more than 25ºC and leaf temperature increased to more than 28ºC. Forsyth & Hall (1965) only treated submerged leaf disks under different temperatures for 10-15 minutes. Therefore, they could not capture developmental changes in leaf structure and physiology under warmer temperatures, as well as stomatal responses to VPD.
Photosynthetic rate could be limited by stomatal conductance over a wide range of environmental conditions (Xu et al. 2016; Verhoef & Egea 2014). However, the negative impact of warming on the photosynthesis of wild blueberries cannot be explained by stomatal limitation as there was no decline in stomatal conductance under warming conditions (Fig. 5d). High leaf-intercellular CO2 concentration under warming conditions also suggests that decline in photosynthesis is not a result of increased stomatal limitation. Instead, the decline in photosynthetic rate (Fig. 5a) and water use efficiency (Fig. 5f) can be explained by the decline in chlorophyll (Fig. 6a & 6b) and nitrogen (N) concentration (Fig. 6c & 6d) which were lower in PH and AH treatments where the air temperature was higher than 25ºC (Fig. 6c & 6d). This implies that physiological performance of the wild blueberry genotype that we studied could decline after reaching the air temperatures of approximately 24 to 25ºC. Leaf nitrogen (N) is one of the most important determinants of photosynthesis (Wright et al. 2004; Reich et al. 1999). Lower leaf N concentration could be because of increased soil water deficits (Fig. 4b) that restrict plant nutrient uptake. Since N is supplied to leaves through the mobile NO3– or NH4+ that are taken up by the roots from the soil mainly through water/mass flow (Barber 1995, Jungk 1996), decreased soil water content can inhibit N uptake. The average Fv/Fm values of the plants which was below 0.8 under warming treatments (both PH and AH) imply that the wild blueberry stems in the AH and PH chambers could be under long-term stress (Maxwell & Johnson 2000).
Warming also changed leaf and stem structural traits. Generally, wild blueberry stems under warming had more leaves that were smaller without changes in total leaf surface area. Stems were smaller, shorter, and with denser wood under warming treatments. All of these suggest higher water deficits under warming treatments. Air humidity (Fig. 3b & 3e), soil water content (Fig. 4b), and leaf water potential (Fig. 4c) were lower under warming. The increased water deficits can decrease cell turgor and consequently leaf and stem development. Additionally, LMA of wild blueberry stems was significantly lower under warming compared to the control. This indicates that leaves were thinner or less dense under warming conditions. High LMA is related to plant adaptation to drought (Gratani et al. 2009, Villar et al. 2013, Zhang et al. 2017). Smaller LMA under warming conditions implies that the wild blueberry plants might not be efficient in enhancing their drought tolerance associated with increased temperatures.
Overall, whole stem CO2 assimilation rate in wild blueberries (Fig. 8) under warming were 32% to 40% lower compared to the control, suggesting warming has negative effects on stem level physiological performance. Declined whole stem CO2 assimilation also suggests that warming may also decrease the yield, which needs to be further studied over a diversity of genotypes.
Conclusion
We suggest by proving in our study that the active heating (AH) open-top chamber is an effective system to study warming effects on wild blueberries in the field. The passive heating chambers, however, introduced some uncertainties including the confounding effects of cooler nights. The AH system successfully manipulated atmospheric warming and can also be applied to other crops like potatoes in Maine. Our results, while preliminary and based upon only one genotype, suggest a potential negative effect of warming on wild blueberry growth by decreasing soil water and nutrient availabilities. Consequently, warming will make summer drought worse, which itself is predicted to increase in frequency and severity in Maine (The University of Maine Climate Institute 2015). Thus, to sustain wild blueberry production in a future with warmer and drier summers, techniques to mitigate these effects should be developed and tested. Potential sustainable solutions, e.g. effective irrigation, mulching, and nutrient management could be tested with the AH system presented here. Our system could also be used to investigate different responses of different wild blueberry genotypes and other crops to climate change. Additionally, our system can be used to study the response of weeds, pathogen disease, pollinators, herbivores, micro-organisms, and fruit quality to warming. Therefore, it could be a platform fostering interdisciplinary research. Those who are interested in studying climate change effects on wild blueberries, other crops, or microorganisms in the field can contact the authors for collaborative research or chamber construction guidelines.
Acknowledgements
We thank Mr. Joshua Stubbs and Christopher McManus from the Blueberry Hill Farm for constructing the chambers and helping with field measurements. We also thank Ailish Scott to help with field measurements. We acknowledge the Maine Agricultural and Forest Experiment Station (MAFES) Analytical Laboratory and Maine Soil Testing Service for leaf sample analysis. This research is supported by Wild Blueberry Commission of Maine, Maine Food and Agriculture Center, Maine Department of Agriculture, Conservation and Forestry, Maine Agricultural and Forest Experiment Station, and USDA National Institute of Food and Agriculture Hatch (ME0-21832).
List of Abbreviations
AH: Active Heating
PH: Passive Heating
OTC: Open-Top Chamber
NC: No Chamber
IPCC: International Panel of Climate Change
LMA: Leaf Mass per Area
LWP: Leaf Water Potential
N: Nitrogen
VPD: Vapor Pressure Deficit
VWC: Volumetric Water Content
References
Badaruddin, M., Reynolds, M.P. & Ageeb, O.A.A. 1999. Wheat management in warm environments: 975-983.
Bajcz, A.W. & Drummond, F.A. 2017. Flower power: Floral and resource manipulations reveal how and why reproductive trade‐offs occur for lowbush blueberry (Vaccinium angustifolium). Ecology and evolution 7(15): 5645-5659.
Barber, S.A. 1995. Soil Nutrient Bioavailability. Wiley, New York.
Bell, D.J., Rowland, L.J., Smagula, J. & Drummond, F.A. 2009. Recent Advances in the Biology and Genetics of Lowbush Blueberry. Maine Agricultural and Forest Experiment Station, University of Maine, Orono. Tech. Bull. 36.
Bita, C. & Gerats, T. 2013. Plant tolerance to high temperature in a changing environment: scientific fundamentals and production of heat stress-tolerant crops. Frontiers in plant science 4: 273.
Bokhorst, S., Huiskes, A., Convey, P., Van Bodegom, P.M. & Aerts, R. 2008. Climate change effects on soil arthropod communities from the Falkland Islands and the Maritime Antarctic. Soil Biology and Biochemistry 40(7): 1547-1556.
Climate Change, IPCC 2014: Synthesis Report. Contribution of Working Groups I, II and III to the Fifth Assessment Report of the Intergovernmental Panel on Climate Change. IPCC.
Curtis, O.F. & Clark, D.G. 1950. An introduction to plant physiology. 1st ed. McGraw Hill Book Conlparry Inc., New York.
Easterling, W.E., Aggarwal, P.K., Batima, P., Brander, K.M., Erda, L., Howden, S.M., Kirilenko, A., Morton, J., Soussana, J.F., Schmidhuber, J. & Tubiello, F.N. 2007. In M.L. Parry et al. (ed.) Climate change 2007: Impacts, adaptation and vulnerability. Contribution of Working Group II to the Fourth Assessment Report of the Intergovernmental Panel on Climate Change. Cambridge Univ. Press, Cambridge, UK. Food, fibre and forest products: 273-313.
Fang, S., Tan, K.Y. & Ren, S. 2010. Winter wheat yields decline with spring higher night temperature by controlled experiments. Scientia Agricultura Sinica 43(15): 3251-3258.
Forsyth, F.R. & Hall, I.V. 1965. Effect of leaf maturity, temperature, carbon dioxide concentration, and light intensity on rate of photosynthesis in clonal lines of the lowbush blueberry, Vaccinium angustifolium Ait. under laboratory conditions. Canadian Journal of Botany 43(8): 893-900.
Gratani, L., Varone, L. & Crescente, M.F. 2009. Photosynthetic activity and water use efficiency of dune species: the influence of air temperature on functioning. Photosynthetica 47(4): 575-585.
Hatfield, J.L., Boote, K.J., Kimball, B.A., Ziska, L., Izaurralde, C., Ort, D., Thomson, A. & Wolfe, D. 2011. Climate impacts on agriculture: Implications for agronomic production. Agronomy Journal 103: 351-370.
Hunt, J.F., Honeycutt, C.W., Starr, G. and Yarborough, D. 2008. Evapotranspiration rates and crop coefficients for lowbush blueberry (Vaccinium angustifolium). International journal of fruit science 8(4): 282-298.
Jungk, A.O. 1996. Dynamics of nutrient movement at the soil-root interface. In Plant Roots-the Hidden Half. 2nd edition. Marcel Dekker, Inc. New York: 529-556.
Lobell, D.B. & Field, C.B. 2007. Global scale climate–crop yield relationships and the impacts of recent warming. Environmental research letters 2(1): 014002.
Magan, N., Medina, A. & Aldred, D. 2011. Possible climate‐change effects on mycotoxin contamination of food crops pre‐and postharvest. Plant Pathology 60(1): 150-163.
Maine’s Climate Future – 2015 Update. The University of Maine Climate Institute 2015. http://climatechange.umaine.edu/research/publications/climate-future
Maxwell, K. & Johnson, G.N. 2000. Chlorophyll fluorescence-a practical guide. Journal of experimental botany 51(345): 659-668.
Mitchell, R.A.C., Lawlor, D.W., Mitchell, V.J., Gibbard, C.L., White, E.M. & Porter, J.R. 1995. Effects of elevated CO2 concentration and increased temperature on winter wheat: Test of ARCWHEAT1 simulation model. Plant Cell Environment 18: 736-748.
Reich, P.B. et al. 1999. Generality of leaf trait relationships: A test across six biomes. Ecology 80: 1955-1969.
Reynolds, M.P., Delgado, M.I., Gutierrez-Rodriguez, M. & Larqué-Saavedra, A. 2000. Photosynthesis of wheat in a warm, irrigated environment. I. Genetic diversity and crop productivity. Field Crops Research 66: 37-50.
Semenov, M.A. & Shewry, P.R. 2011. Modelling predicts that heat stress, not drought, will increase vulnerability of wheat in Europe. Scientific reports 1: 66.
Sun, S.Q., Peng, L., Wang, G.X., Wu, Y.H., Zhou, J., Bing, H.J., Yu, D. & Luo, J. 2013. An improved open-top chamber warming system for global change research. Silva Fenn 47: 960.
Verhoef, A. & Egea, G. 2014. Modeling plant transpiration under limited soil water: Comparison of different plant and soil hydraulic parameterizations and preliminary implications for their use in land surface models. Agricultural and Forest Meteorology 191: 22-32.
Villar, R., Ruiz‐Robleto, J., Ubera, J.L. & Poorter, H. 2013. Exploring variation in leaf mass per area (LMA) from leaf to cell: an anatomical analysis of 26 woody species. American Journal of Botany 100(10): 1969-1980.
Wright, I.J. et al. 2004. The worldwide leaf economics spectrum. Nature 428: 821–827.
Xiao, G., Zhang, Q., Li, Y., Wang, R., Yao, Y., Zhao, H. & Bai, H. 2010. Impact of temperature increase on yield of winter wheat at low and high altitudes in semiarid northwestern China. Agricultural Water Management 97: 1360-1364.
Xu, X., Medvigy, D., Powers, J.S., Becknell, J.M. & Guan, K. 2016. Diversity in plant hydraulic traits explains seasonal and inter-annual variations of vegetation dynamics in seasonally dry tropical forests. New Phytologist 212: 80-95.
Zhang, Y.J., Cristiano, P.M., Zhang, Y.F., Campanello, P.I., Tan, Z.H., Zhang, Y.P., Cao, K.F. & Goldstein, G. 2016. Carbon economy of subtropical forests. In Tropical Tree Physiology Springer, Cham. 337-355.
Zhang, Y.J., Sack, L., Cao, K.F., Wei, X.M. & Li, N. 2017. Speed versus endurance tradeoff in plants: Leaves with higher photosynthetic rates show stronger seasonal declines. Scientific reports 7: 42085.